Electron beam is a versatile and sustainable technology that, in the RadTech sphere, generally is discussed as a way of reducing CO2 emissions through the elimination of solvents and reduction in energy consumption for ink, coating and adhesive applications. Yet, it also may provide a solution for CO2 production when emissions are not as easily avoided. Researchers at GTI Energy are studying the possibility of using EB to transform CO2 into valuable products.
As an industrialized nation, the US produces a large amount of greenhouse gas emissions – approximately 6,300 million metric tons CO2 equivalents in 2022, according to the EPA.1 Almost 50% of those emissions are the result of energy production or industry. 2 Left untreated, the exhaust from a coal-fired power plant, for example, can contain as much as 300 times the amount of CO2 as the earth’s atmosphere.3 These carbon emissions then end up in one of three areas. About 25% is captured by vegetation, such as grasslands, bogs and forests. 4 Another 45% is held in the atmosphere. The remaining 30% is absorbed in the surface layer of the oceans, raising the water’s acidity.
What to Do with All the CO2?
In combination with reducing CO2 emissions, carbon capture and sequestration can be utilized to prevent excess carbon in the atmosphere and oceans. Biological sequestration can be increased by restoring wetlands, planting trees and improving agricultural practices, while preserving existing natural environments. Sometimes considered the simplest solution and the easiest to implement, biological sequestration on a large scale requires cooperation, knowledge, proper planning, space and long-term dedication. However, it alone likely is not sufficient.
The next category of carbon capture and sequestration is geological sequestration, which often is referred to as carbon capture and storage. Geological sequestration is the process of storing CO2 emissions deep underground in geological formations. It is a controversial practice for several reasons. Carbon pipelines are needed to transport the emissions to the storage location, and they, like other pipelines, raise questions of eminent domain and leakage safety concerns. If the carbon is not utilized, only stored, the storage infrastructure, operation and maintenance costs are not offset in any way and are expected to be passed on to the consumer, raising the price of electricity, for example. Additionally, storage capacity is not unlimited, nor is it equally distributed across the globe. For instance, the US has an estimated capacity of 3,900 gigatons of carbon dioxide (GtCO2), whereas eastern Europe only has ~10% that capacity. 5 The estimated global capacity is ~11,000 GtCO2 – predicted to last only the next century.
The third and final category of carbon capture and sequestration is technological sequestration, a broad category that encompasses the many ways technology can be used to contain carbon emissions. When technology is employed to transform those emissions into useful products (e.g., graphene), technological sequestration also is known as carbon capture and utilization. A benefit of carbon utilization is that often useful products are potentially profitable. New technologies also constantly are being developed or turned toward the problem of CO2, including electron beam.
EB for Carbon Utilization
Researchers at GTI Energy recognized EB as an energy efficient method of chemical transformation and investigated its ability to turn CO2 into synthesis gas (syngas). 6 Syngas is a combination of carbon monoxide and hydrogen (CO:H2, ratios vary) that is a substitute for fossil fuels, including natural gas, in electricity or heat production. It also is a feedstock for the chemical synthesis of a wide range of chemicals, such as hydrogen, methanol and ammonia.
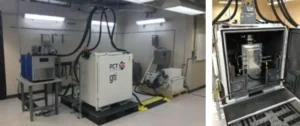
GTI worked with PCT Ebeam and Integration to custom-design a lab-scale 200 kV EB unit for gas flow (Figure 1). 6 Gases were mixed outside of the EB unit, flowed in front of the beam, and then products were collected and tested. GTI researchers determined that syngas could be synthesized by electron beam by combining carbon dioxide and methane (Equation 1) at moderate temperatures (<150° C) and near atmospheric pressure without a catalyst.
In its work High-Energy Systems for Transforming CO2 into Valuable Products, GTI investigated ways of optimizing the EB synthesis reaction by varying different parameters. 6 In one such example, it experimented with the composition of the feed gas and found a 0.5 mol fraction of CO2 produced the greatest conversion (Figure 2).
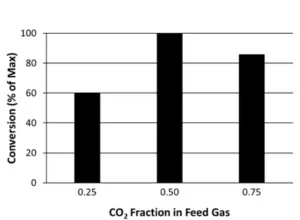
GTI also varied the power of the EB system and recorded its effect on the product (measured as CO) concentration (Figure 3, A).6 The CO concentration reached a maximum at 0.9 kW beam power, then decreased at higher power levels. EB power (kW, where kW = kV*A) can be increased in two ways – increasing the accelerating voltage (kV) or the beam current (A). When the accelerating voltage is increased, greater penetration of energy through the gas is achieved (Figure 3, B), and the concentration of product also would be expected to increase (until the beam penetration exceeds the reactor depth).
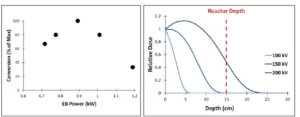
Increasing the beam current (which is proportional to increasing dose for a given gas flow rate) does not always lead to as straightforward of results and may be the reason for the decrease in conversion at 1 and 1.2 kW. There is a dose level in almost every application where destruction overtakes formation. In this case, since CO was the
only measured product, other reactions may have occurred at the higher dose levels that did not result in CO formation.
In order to put the EB synthesis of syngas in perspective, GTI chose to compare the method to one of the most popular existing methods of syngas production – steam methane reforming (SMR). 6 As the name suggests, the process combines steam and methane as reactants (Equation 2).
SMR is a reaction driven by natural gas combustion. It requires high temperatures (700-900° C) and pressures (1.5-3 MPa) and a catalyst. The need for natural gas fuel and water negatively impact the global warming potential of SMR. In contrast, the EB method utilizes electricity and does not require water as a reactant. Although more sustainable sources of methane are possible (e.g., biomass), the methane feedstock in both methods is assumed to be from natural gas, which is typical for the SMR process. The results of the lifecycle analysis, using the CO product as a basis for comparison, show EB synthesis has a 60% smaller global warming potential than SMR (Figure 4).
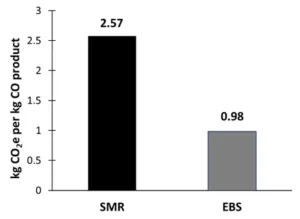
In summary, technology may play a pivotal role in a sustainable future – not only in preventing emissions but also as a way to transform greenhouse gas emissions into valuable products. GTI has shown EB has promise as one of these technologies, transforming CO2 into syngas.
References
- EPA, Inventory of US Greenhouse Gas Emissions and Sinks. https://www.epa.gov/ghgemissions/inventory-us-greenhouse-gas-emissions-and-sinks#:~:text=Key%20findings%20from%20the%20latest,sequestration%20from%20the%20land%20sector.
- EPA, Sources of Greenhouse Gas Emissions. https://www.epa.gov/ghgemissions/sources-greenhouse-gas-emissions
- Moseman, A., How Efficient is Carbon Capture and Storage? 2021. https://climate.mit.edu/ask-mit/how-efficient-carbon-capture-and-storage#:~:text=Consider%20that%20untreated%20exhaust%20from,still%20leaves%20a%20lot%20behind
- Bennedsen, M., Hillebrand, E., Koopman, S., “Trend analysis of the airborne fraction and sink rate of anthropogenically released CO2.” Biogeosciences. 16 (18): 3651–3663. 2019.
- Dooley, JJ., et. al., Carbon Dioxide Capture and Geologic Storage: Core Element of a Global Energy Technology Strategy to Address Climate Change. 2006. https://www.epa.gov/sites/default/files/2015-05/documents/act_2007_02_battelle.pdf
- Akpolat, O., et. al., High Energy Systems for Transforming CO2 to Valuable Products, 2022. https://www.osti.gov/biblio/1887520
Sage Schissel, Ph.D.
Applications Specialist
PCT Ebeam and Integration LLC
sage.schissel@pctebi.com