By Sam Garton and Neil Cramer, Colorado Photopolymer Solutions; Victor Piñón III and Ana B. Baca, Sandia National Laboratories; Freddie Santiago, Naval Research Laboratory; and Anthony Coltson, US Food and Drug Administration
Abstract
Glass and polymer optics are used extensively in a variety of applications. Some uses for optics include medical disposables, bar-code scan/recognition, security and fingerprint scanners, motion and presence sensors, rifle scopes, eyeglasses and charge-coupled device (CCD) cameras. Polymer optics offer several advantages over traditional glass optics including lighter weight, ease of manufacturing and lower material cost. Due to these advantages, optical glass materials have been successfully replaced with polymer optics in many applications such as eyeglasses, phone screens and cameras. Current drawbacks of polymer optics include the lack of a high precision fabrication method as well as limited materials that exhibit equivalent material properties to those of glass optics1. There are an abundance of optical glass materials available for use in commercial optical systems and very limited optical polymer materials. In this study, we explore a variety of materials with a wide variation of refractive indices and Abbe numbers to create a broader range of optical properties from which to choose when designing polymer lenses. A plethora of different chemistries utilizing a comprehensive list of monomers will be investigated to create an expansive polymer materials library for use in lens fabrication. The goal is to create polymer optical materials for direct view sighting systems due to their lighter weight, lower material cost and ease of manufacturing.
Introduction
Polymer optics are not widely used due to their inability to obtain optical glass quality and physical properties from traditional chemistries. To increase the availability of polymer optics, step growth polymer reactions were used to form optics while minimizing aberrations formed from shrinkage and stress during the polymerization. In this study, we focus on UV cured thiol-acrylate polymerization systems.
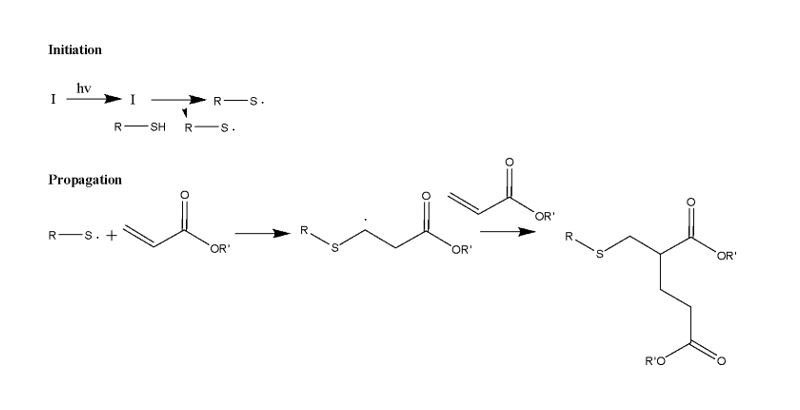
Background
When designing optical systems, two important parameters are Abbe number and birefringence. The Abbe number is the measurement of the material’s dispersion. The higher the dispersion, the lower the Abbe number. Abbe numbers range from 17 to 95 for moldable glasses. Abbe numbers lower than 55 are known as flint glass and have low dispersion while glasses with Abbe numbers higher than 55 are known as crown glass and have high dispersion. Both low and high Abbe numbers are needed for different commercial uses. Birefringence is the variation of refractive index with the polarized state of light. Low birefringence is preferred because this means the lens is uniform and has little variation throughout its surface. Birefringence is mostly induced by the injection molding process, which is a characteristic that UV curing may substantially reduce1.
Chemistry Overview
An extensive list of monomers was created as a starting point to obtain a wide range of refractive index and Abbe numbers for the polymer optics. Once the monomers were selected, Table 1 was constructed to summarize the pros and cons of each chemistry type being investigated. Figures 1 through 4 illustrate the mechanism for these chemistries.
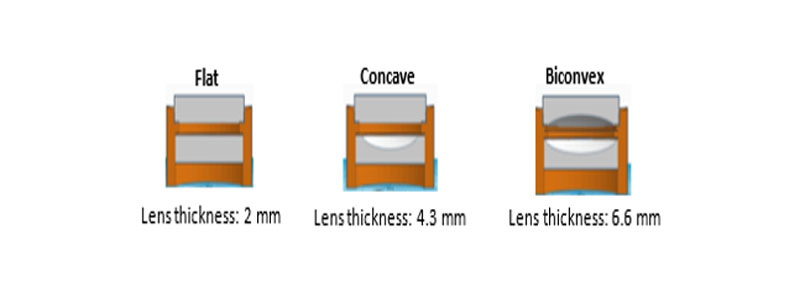
Molding Procedures
Utilizing thiol-ene and thiol-acrylate UV-curable systems allowed for screening of materials to obtain both crown and flint-like polymer optics in a variety of configurations. Molds were custom 3D printed and designed to exhibit good flexibility to allow the mold to shrink with the polymer as it cured. This reduced the optical aberrations at the lens surface when removing the lens from the molds. After curing, the lenses were demolded by swelling the mold in isopropyl alcohol for 30 minutes. Figure 2 shows the various mold configurations that were custom 3D printed.
In addition to flat, concave and biconvex lenses, doublets also were made, as these are important in many applications due to their increase in focus. Figure 3 shows this shift in focus.
Although aspherical lenses were not fabricated in this study, they are important in the industry because they converge the focal point of the lens. Figure 4 shows this convergence of the focal point.
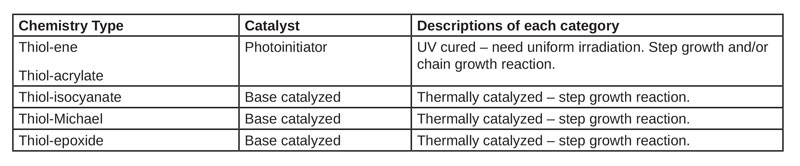

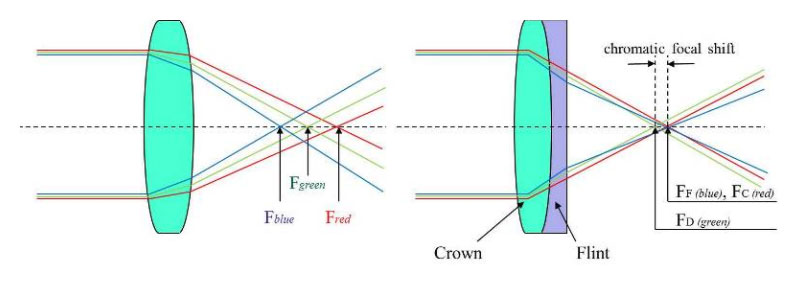
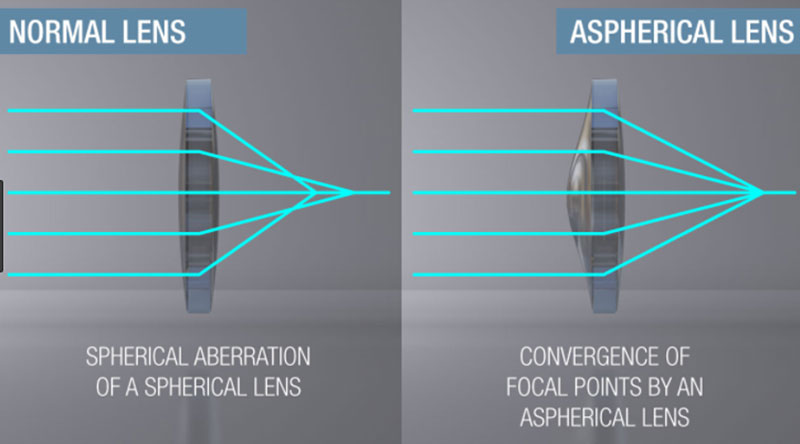
Curing Procedures
Upon initial curing of the molds, it was evident that uneven and rapid curing was causing poor optical quality. Using a thermocouple, the resin temperature was shown to rise by 30°C in the first 5 seconds of curing and rose over 100°C within 20 seconds. Thermal imaging was used to monitor whether the heat evolution was uniform or if hot spots were seen. Figure 5 shows hot spots throughout the mold.
To reduce the rate of the polymerization, less photoinitiator and lower intensity lights were used along with adding a neutral density filter to further drop the intensity. The photoinitiator concentration was reduced from 0.5 weight percent to 0.01 weight percent and the light intensity was reduced from 5 mW/cm2 to 150 µW/cm2. It was found that a distance of 20 cm from the lens to the light source was optimum for the most uniform cure. A light box was used to obtain a uniform cure throughout the lens. Figure 6 shows the optimum setup to obtain high quality polymer lenses.
Results
Table 2 shows two different thiol-acrylate UV-curable lens formulations that yielded optical quality glass in both crown and flint materials. These materials were molded using a flat custom 3D-printed mold and cured in the method explained above. CPS 2 was chosen to be made in several different configurations due to its low wavefront error. Figures 7 and 8 show CPS 2 made in a flat configuration.
Using Zygo laser interferometry, the fabricated lenses were analyzed for their peak-to-valley height (PV), root-mean-square (RMS) value and power. A wavefront map of the results can be seen in Figure 9. The PV value indicates how much the lens surface deviates from an ideal, perfectly smooth surface, and RMS is the average of PV values taken from a lens. A summary of the results for different lenses is given in Table 3.

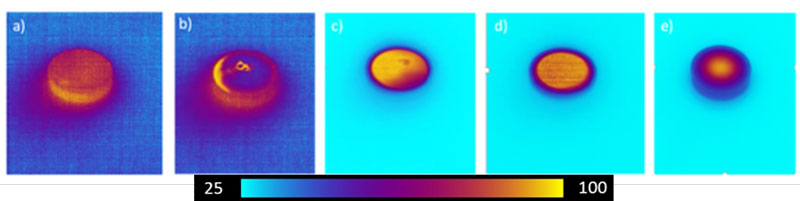
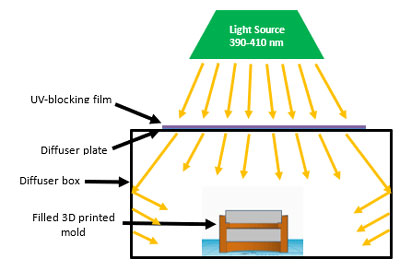
As seen in Table 3 the CPS 2 has a PV value in the flat configuration of 1.45 compared to a glass mold of 0.3. A PV value less than 2 is considered substantial and a PV value less than 1 is considered good optical quality glass. The curing optimizations described in this work have led to the PV value of the polymer optics decreasing substantially. Continued improvements are anticipated.
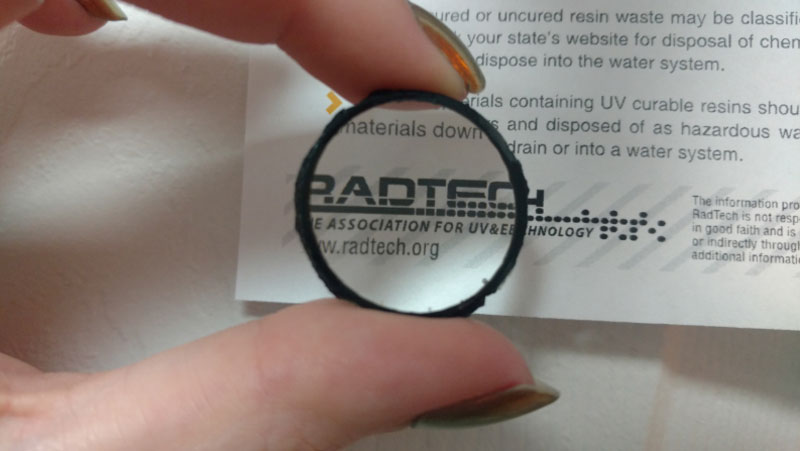
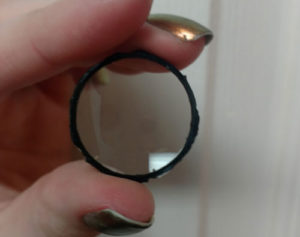
Conclusion
Utilizing UV-curable polymers to create optics has several advantages over traditional glass optics. The minutes needed to mold a polymer optic, compared to the days needed to produce a commercial glass optic – in conjunction with the low-cost setup of UV curing – certainly makes it a desirable manufacturing process. While the optical quality of the polymer optics is quite good, additional progress is needed to fabricate high-quality optical polymers.
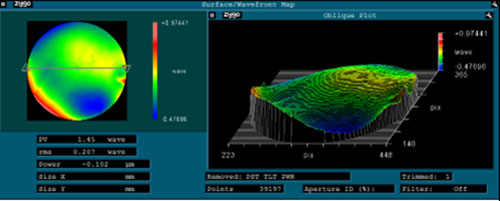
Remarks from Sandia National Laboratories
This paper describes objective technical results and analysis. Any subjective views or opinions that might be expressed in the paper do not necessarily represent the views of the US Department of Energy or the United States Government.
Sandia National Laboratories is a multimission laboratory managed and operated by National Technology & Engineering Solutions of Sandia, LLC, a wholly owned subsidiary of Honeywell International Inc., for the US Department of Energy’s National Nuclear Security Administration under contract DE-NA0003525.
References
- Symmons, Alan, and Michael P. Schaub. Field Guide to Molded Optics.
- Cramer, Neil B., and Christopher N. Bowman. “Kinetics of Thiol-Ene and Thiol-Acrylate Photopolymerizations with Real-Time Fourier Transform Infrared.” Journal of Polymer Science Part A: Polymer Chemistry, Vol. 39, No. 19, 2001, pp. 3311–3319., doi:10.1002/pola.1314.
- Cramer, Neil B., et al. “Mechanism and Modeling of a Thiol-Ene Photopolymerization.” Macromolecules, Vol. 36, No. 12, 2003, pp. 4631–4636., doi:10.1021/ma034072x.
- Piñón, Victor, and Freddie Santiago. “UV Cured Polymer Optics.” Optifab Manuscript 2017.