The most common allotropic form of the element Carbon is graphite. This inorganic polymeric material is found in all pencils, where it mistakenly is called “lead.” Despite being a non-metal, it also is an excellent conductor of electricity and is used as an electrode in laboratory and industrial settings. The usefulness of graphite in pencils comes from the fact that its molecular structure consists of two-dimensional (2D) flat layers of carbon stacked on top of one another. These layers of carbon atoms have strong, alternating covalent double bonds among the atoms within each layer, but very weak Van der Waals attraction between adjacent layers. This weak attraction makes it easy for the layers to slip past one another, allowing them to be removed easily from the stack with simple friction on paper. This same structural reality makes graphite a very useful industrial lubricant. Graphite’s electrical conductivity comes from the fact that each individual 2D layer consists of six-membered hexagonal carbon rings fused together with an abundance of delocalized p-electrons that can move easily throughout the sheet when an external voltage is applied.
In 2004, Andre Geim and Konstantin Novoselov of the University of Manchester produced a single monatomic layer of graphite, creating what is known as “graphene” in the process. 1 Even earlier than that, in 1991, Sumio Iijima of Japan prepared the first carbon nanotubes (CNTs). 2 These are tubes of pure carbon derived from rolling graphene sheets into cylindrical tubes with diameters of several nanometers (nm). Single-walled carbon nanotubes (SWNTs) consist of a single layer of carbon atoms, while multiwalled carbon nanotubes (MWNTs) consist of several concentric tubes. The size and properties of MWNTs, as one might guess, significantly are different from those of SWNTs.
Since their development, these materials have added greatly to the field of materials science. Kumar reported in December 2003 that approximately 7,500 research papers were published on the use of CNTs up to that time. 3 That work has continued unabated, with the American Chemical Society stating in a 2021 article in the journal ACS Nano that, following the report by Iijima, the number of published papers significantly increased each year, leveling off at ~8,000 per year after 2010, 4 including more than 1,000 CNT papers published in ACS Nano itself.
With this incredible interest in CNT utilization, it is appropriate to ask what property enhancements one might discover from incorporation of CNTs in UV-polymerizable formulations. In the early 2000s, students at the University of Houston-Downtown began experimenting with CNTs as additives in UV-polymerizable formulations. This work began with SWNTs, but MWNTs also were later investigated. The results of these studies were presented at RadTech conferences in 2006 and 2008, respectively. 5, 6 The purpose of this article is to review and summarize that work.
Preparation of UV-Polymerizable, SWNT-Containing Formulations
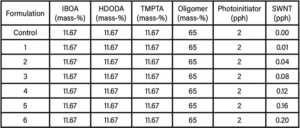
The first part of this study involved incorporating SWNTs into UV-polymerizable formulations based on an acrylate-functional aliphatic urethane oligomer. This oligomer was blended with three acrylate-functional monomers: isobornyl acrylate (IBOA), 1,6-hexanediol diacrylate (HDODA),and trimethylolpropane triacrylate (TMPTA), along with the photoinitiator 1-hydroxycyclohexylphenyl ketone. Added to this control formulation were SWNTs ranging from 0.01-0.20 parts per hundred (pph) of the oligomer/monomer mixture. These seven formulations are shown in Table 1.
To expect to see the full benefit of SWNTs in UV-polymerizable formulations, it is assumed that the SWNTs need to be exfoliated from one another. These are non-polar materials, but due to the large number of delocalized p-electrons, SWNTs are very polarizable! That means that polar molecules, such as acrylates, can induce dipoles in the SWNTs, creating in the process dipole-induced dipole interactions. 7,8,9 This implies that dispersing the nanotubes in acrylate-functional monomers and oligomers should be relatively easy, precluding the need for surfactants or functionalization of the nanotubes themselves. In the work at UH-Downtown, this was found to be the case. However, because of the viscosity of the oligomer, it was necessary to disperse the SWNTs systematically in the monomer mixture using sonication techniques prior to adding the highly viscous oligomer (a procedure that involved a total of nine hours of sonication 5).
Experimental Demonstration of Exfoliation
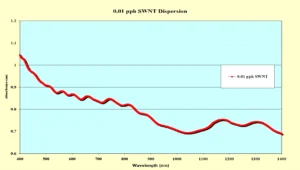
The resulting SWNT dispersions gave the appearance of a uniform mixture that seemed to be stable with respect to phase separation and settling. But, to further determine the effectiveness of this dispersion method, Visible/Near Infrared (Vis/NIR) spectroscopy and viscosity measurements were utilized. Figure 1 shows the Vis/NIR spectrum of the sample containing 0.01 pph SWNT. The “ripples” seen in the spectrum are known as van Hove singularities. They demonstrate the presence of individual, well-exfoliated SWNTs in the formulation. 10 Further verification of exfoliation involved studying the rheology of each formulation. A Brookfield Model DV111 Programmable Rheometer with a TC500 Temperature Control Bath was used to study the effect of the concentration of SWNTs on the rheological properties of the dispersions. The temperature of each sample was held to 25.0° C and a #25 cylindrical spindle was utilized. Figure 2 shows a graph of viscosity vs. shear rate for each of the seven formulations. Figure 3 shows an enlarged section of Figure 2 for the formulations containing 0.00-0.08 pph SWNT.
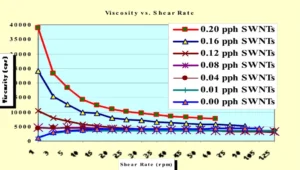
The first thing to note from these two graphs is that at 1 rpm shear rate, the viscosity of the formulation increases with increases in the SWNT concentration. This indicates good interaction among the nanotubes and the acrylate-functional components and, thus, provides further evidence of effective exfoliation of the SWNTs. Next, the control formulation and the 0.01 pph SWNT sample each show “shear thickening” (dilatant) behavior up to about 15 to 20 rpm, at which point they become essentially Newtonian. This effect is even more evident in Figure 3. This behavior may indicate when the blends are subjected to shear forces, the component molecules realign to give more intimate contact, increasing the viscosity up to a limiting value, above which the rheology becomes Newtonian. Perhaps even more interesting is the fact that the 0.01 pph sample has an ultimate viscosity that is significantly lower than that of the control formulation and is lower at all shear rates investigated except for the reading at 1 rpm. Furthermore, above 20 rpm shear rate, the 0.04 and 0.08 samples also have viscosities lower than that of the control. In other words, they are “shear thinning” (pseudoplastic). This indicates that the relatively strong intermolecular attractive forces within the control sample are somewhat disrupted by the introduction of SWNTs into the formulation. Finally, note that the remaining three formulations also are shear thinning. One can see in Figure 2 that the higher the concentration of SWNTs, the greater the pseudoplastic behavior of the samples. This shear thinning behavior indicates that in the absence of shear, the four higher concentration samples have a higher resistance to flow. Thus, while the interactions among the monomers, the oligomer and the SWNTs can produce good dispersion, the network is somewhat broken down under relatively low shear conditions.

In Part 2 of this article, the effects of SWNTs on the reactivity of the formulations will be discussed, along with the effects they have on the thermal and thermomechanical properties of the formulations, including effects on the glass transition temperature (Tg).
Byron K. Christmas, Ph.D.
Professor of Chemistry, Emeritus
University of Houston-Downtown
b4christmas@gmail.com
References
- https://www.graphene.manchester.ac.uk/learn/discovery-of-graphene/ – accessed June 14, 2023.
- S. Iijima & T. Ichihashi, Nature, 363 (1993) 603.
- S. Kumar, “Polymer/Carbon Nanotube Composites: Opportunites and Challenges”, International Symposium on Nanostructured Polymeric Materials, Tokyo, Japan, December 4-5, 2003.
- Yan Li, “Carbon Nanotube Research in Its 30th Year”, ACS Nano 2021 15 (6), 9197-9200.
- V.Lam, G. Patino, C. Carandang, & B. Christmas, “UV-Polymerizable Systems Containing Single-Walled Carbon Nanotubes,” http://www.radtech.org/proceedings/2006/papers/093.pdf – Accessed June
16, 2023. - N. Lam, D. Kurukji, S. Luce, & B. Christmas, “Acrylate-Functional Urethane- and Polyester-Based Formulations Containing Multi-Walled Carbon Nanotubes,”
http://www.radtech.org/proceedings/2008/papers/113.pdf – Accessed June 16, 2023. - B. Christmas, “Intermolecular Attractive Forces: The Key to Understanding Polymer Properties-Part 1”, UV+EB Technology, Vol.7, No. 4, Quarter 4, 2021, pp. 16-17.
- B. Christmas, “Intermolecular Attractive Forces: The Key to Understanding Polymer Properties, Part 2”, UV+EB Technology, Vol. 8, No. 1, Quarter 1, 2022, pp. 18-19.
- B. Christmas, “Intermolecular Attractive Forces: The Key to Understanding Polymer Properties, Part 3”, UV+EB Technology, Vol. 8, No. 2, Quarter 2, 2022, pp. 12-13.
- G. Lanzani & L. Lüer, “Carbon Nanotubes: Electronic Structure and Spectroscopy”, Comprehensive Nanoscience and Technology, Vol. 1, 2011, pp. 23-39.