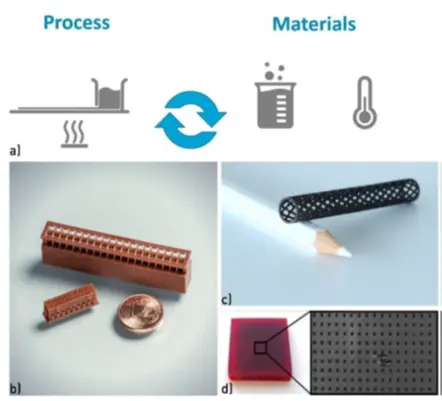
By Christian Frank, Kristina Hager, Reka Csizmadia, Markus Kury and Christian Gorsche, Cubicure GmbH
Additive manufacturing (AM) has gained wide acceptance in R&D departments of companies in major industries (e.g., mobility, electronics, medical, aerospace, tooling) and their strategic initiatives toward industrial production solutions greatly contribute to the AM industry’s recent upswing. Global sales of AM products and services showed double-digit growth rates from 1990 to 2020, highlighting this great potential. From the most prestigious AM industry annual report (Wohler’s Report 20201), photopolymers (from resin-based 3D-printing processes) occupy the most important class of materials in the AM market, accounting for more than 30% of total material-related sales. Above all, however, photopolymers also already are being used in AM-based industrial production (e.g., for personalized orthodontic splints or hearing aid shells), although here still exclusively as molded samples or for components subjected to little mechanical stress. The technical use of AM components primarily as a product development solution in the performance evaluation of later-series parts represents the most promising entry of AM polymers into industrial part production, yet it cannot be successfully implemented at the present stage due to a lack of either 1) part quality or 2) material performance of the available AM components.
Resin-based stereolithography (SLA), which can achieve very high surface quality (Ra < 10 μm), is an excellent candidate for the production of industrial-grade polymer components. SLA uses light-curable resin formulations that are cured layer-by-layer using either a laser or a digital projector, which allows polymers to be fabricated with excellent precision.2
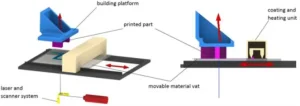
However, the SLA materials currently available on the market are not particularly suited for industrial use as functional components, as they tend to exhibit brittle material behavior and are lacking the necessary functional properties (e.g., flame resistance, long-term stability).
Cubicure GmbH developed and patented a new SLA process, Hot Lithography® (Figure 1). The concept behind it is to take advantage of a light-based SLA technology and provide solutions to the associated material obstacles. In order to introduce more functionality (e.g., toughness, heat deflection temperature, durability, flame resistance) to SLA materials, a number of parameters need to be addressed, but these changes typically lead to challenges related to processing of the respective resin formulations. Reducing the cross-link density of the photopolymer network via use of high molecular weight or oligomeric derivatives, introducing intermolecular forces into the resin backbone (e.g., urethane groups) or the option to process composites (e.g., organic or inorganic fillers), for example, are strategies that have a positive impact on the final performance of the material but have dramatic effects on the reactivity and resin viscosity of the light-curing systems.
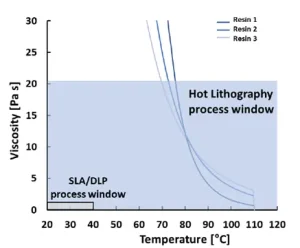
Resin optimizations that could lead to higher material performance (e.g., toughness or heat deflection temperature) and smoother processing (e.g., reduction of polymerization shrinkage) are limited severely in standard lithography-based AM technologies due to a rather narrow processing window (e.g., viscosities < 2 Pa s and processing temperatures < 60°C). These limitations are overcome with the technology described in this article by enabling higher temperatures during the printing process (up to 120°C) and opening the process to higher resin viscosities (up to 20 Pa s at processing temperature). Thus, this new SLA process offers unique opportunities for material development by expanding the processing window for new material solutions and 3D printable resin formulations (Figure 2).
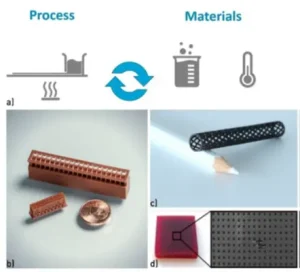
Thus, the new patented process makes it possible to develop functional AM photopolymers and, from them, AM components with high potential for industrial applications. This ideally is realized through a strong interaction between the AM process and material development. Already successfully developed tough AM photopolymers (e.g., combined tensile strength > 40 MPa, elongation at break > 15% and heat deflection temperature > 70°C) with additional high surface quality of the AM components (e.g., resolution of 10 to 50 μm depending on laser spot and layer height, roughness Ra < 10 μm) and additional key properties, like flame resistance, show the high potential of this AM technology (Figure 3).
Polymer part manufacturing for the electronics industry needs to support specific material performance, high manufacturing precision and surface quality. Clearly, lithography-based AM technologies (e.g., SLA, DLP) offer such critical performance characteristics, yet available materials still fail to support industrial expectations. In this paper, methacrylate-based photopolymers with flame-retardant additives are studied for their suitability to print flame-retardant photopolymers via the new patented process.
Materials and Methods
Methacrylate-based photopolymers with flame-retardant additives
Formulations and sample preparation
As base resin formulation, referenced as Matrix, the commercial resin Evolution provided by the authoring company was used. As flame-retardant additives, established derivatives from the polymer industry were selected (i.e., FR1 – diphenyltolylphosphat, a liquid phosphorous-based derivative; FR2 – bis(2-ethylhexyl)-3,4,5,6-tetrabromophthalate, a liquid, halogen-based derivative; FR3 – a liquid, oligomeric phosphorous-based derivative; FR4 – aluminium hydroxide, a solid, inorganic mineral filler; and FR5 – a solid, phosphorous-based intumescent derivative). The flame retardants FR1 and FR2 were purchased from Sigma Aldrich and FR3 through 5 were kindly provided by the LKT – Laboratorium für Kunststofftechnik GmbH in Vienna, Austria. All flame-retardant additives were mixed homogeneously with the Matrix at 70°C and in the respective contents by weight (e.g., 10 wt% FR3 for sample FR3_10). For the horizontal burning tests, rectangular specimens with the measurements 25 x 4 x 2 mm3 were molded in a UV chamber from Uvitron International, Intelliray 600. The used electromagnetic energy ranged from visible to UV light from 250 to 600 nm with an intensity of 178.8 mW/cm2 (100%) and samples were cured for 2×5 min and flipped in between irradiation cycles.
3D printing via new patented process for preparation of (thermo)mechanical test specimens
Specimens for the vertical burning test (125 x 12.7 x 3 mm3), the heat-deflection temperature tests and tensile tests were printed from respective resin formulations on a commercial Caligma 200 printer by the authoring company with a layer height of 100 μm. The Caligma printer is set up with a 405 nm diode laser source. The laser beam is scanned over a 2D plane using a galvanometer scanning system. All parts that had direct contact with the formulation were heated to 60° C to 80°C; this includes the material vat, the building platform and the recoating unit. The laser spot on the surface of the material vat has a diameter of ~18 μm (FWHM). The printed samples were post-processed by cleaning with isopropanol and ensuing thermal curing at 120°C for 2 h and then 150°C for 2 h in a Carbolite Gero LHT oven. Prior to (thermo)mechanical testing, all specimens were placed in a conditioning box for a minimum of 88 h at 23°C and 50% relative humidity.
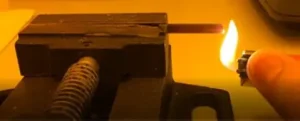
Horizontal and vertical burning tests
For a first assessment on flammability, the molded photopolymers were clamped horizontally and flamed with a lighter for 5 s. Then the time until the flame self-extinguished was detected or the photopolymers completely burned down (Figure 4). The vertical burning tests on the 3D printed specimens were performed in accordance with UL 94 standard at the LKT – Laboratorium für Kunststofftechnik GmbH in Vienna, Austria.
Heat Deflection Temperature (HDT)
Heat deflection temperature (HDT) measurements were performed on an HDT/Vicat 3-300 standard. HDT(B) tests were performed according to DIN EN ISO 75. The sample specimens (with a rectangular geometry of 80 x 10 x 4 mm3) were tested flatwise with a loading force of 0.45 MPa for HDT(B). The starting temperature for each measurement was set to 26°C, and the samples were subjected to the respective loading force for 5 min. Then, a temperature ramp of 120 K min-1 was performed.
Tensile Tests
A ProLine Z010 TH material tester from Zwick/Roell was used for tensile tests of the 3D-printed specimens. The test was performed according to DIN EN ISO 527-2 using 5A specimens. The Young’s modulus was measured within 0.05 to 0.25% of elongation at a strain rate of 1 mm min-1 and then the measurement was continued with a strain rate of 10 mm min-1. Five specimens were tested per sample.
Results and Discussion
Methacrylate-based photopolymers with flame-retardant additives
Herein, the influence of flame-retardant additives on the flammability and on the final (thermo)mechanical properties of 3D-printed photopolymers are investigated. A base resin formulation (Matrix – resin formulation Evolution provided by the authoring company), together with five different flame-retardant additives, was studied. The flame-retardant additives differed in their aggregate state and in their mode of action (FR1 – liquid phosphorous-based; FR2 – liquid, halogen-based; FR3 – liquid, oligomeric phosphorous-based; FR4 – solid, inorganic mineral filler; FR5 – solid, intumescent).
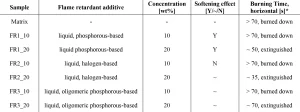
Flammability testing and (thermo)mechanical properties of flame-retardant photopolymers
Photopolymers from the Matrix and from formulations with 10 and 20 wt% added flame-retardant additives were molded in a UV chamber and subsequent horizontal burning tests were conducted on the resulting rectangular photopolymers. The flame-retardant properties successfully were improved by the liquid flame retardants FR1-3 already at 20 wt% contents (Table 1). Whereas the photopolymerized base resin (Matrix) and all samples containing 10 wt% flame-retardant additives burned down completely in the horizontal burning test, the flame extinguished in the burning tests with respective photopolymers containing 20 wt% flame-retardant additives. With the solid flame-retardant additives FR4 and FR5, no significant reduction in flammability was detected for contents up to 20 wt%. All photopolymerized samples also were assessed manually with respect to their mechanical properties and a potential softening effect via the incorporation of flame-retardant additives was recorded. The liquid, phosphorous-based FR1 showed significant softening, which is not desired in the final photopolymer; and the liquid, halogen-based FR2 is not desired due to regulatory restrictions for such compounds. Thus, the liquid, oligomeric phosphorous-based FR3 overall showed the best performance with improved flame resistance, lower impact on mechanical properties and good stability and printability of the final resin.
Subsequently, a combination of FR3 with the solid FRs (i.e., FR4 – solid, inorganic mineral filler; FR5 – solid, intumescent) was tested for potential synergistic effects. In the horizontal burning tests, those photopolymers performed significantly better, thus vertical burning tests also have been performed. For the vertical burning tests, the photopolymer specimens were 3D printed from the prepared resin formulations.
The base resin formulation has a viscosity of ~ 2-4 Pa s at printing temperature, which typically is set to 60° to 80°C. The investigated liquid flame-retardant additive FR3 is soluble in the resin formulation, and resin viscosity was not impacted significantly. The solid flame-retardant additives FR4 and FR5 led to an increase in viscosity, which meant that process parameters had to be adjusted to realize a reliable printing process. In terms of curing depth and print resolution, the liquid flame retardant showed no impairment, the solid additives reduced curing depth and caused significant overpolymerization due to scattered light. As those solids both scatter and absorb UV, formulations ideally could be adjusted using processing additives, such as light absorbers. Furthermore, while the liquid flame retardant did not result in any visual or surface effects on the final 3D parts, the addition of solid flame retardants leads to a rougher surface texture, dependent on the implemented filler particles.

Synergistic effects could be detected with the liquid/solid combination FR3/FR5_20/20, which even passed the vertical burning tests performed in accordance with UL 94 with 3 mm-thick samples (Table 2). This result indicates that such a material concept could pass the industrially relevant UL 94 testing, which is a critical requirement for the electronics industry.

In addition to the vertical burning tests, tensile bars and specimens for the assessment of heat deflection temperature were 3D printed as well. Figures 5 and 6 display the influence of the implemented flame-retardant additives on the (thermo)mechanical properties of the resulting photopolymers. The green properties or “as printed” (see described softening effect in Table 1) were reduced significantly by all liquid flame-retardant additives FR1 through 3. Thus, higher proportions of the liquid flame retardants were not feasible, since at 20 wt% FR-content the final tensile strength post-processing was reduced by > 20 to 60% and the Young’s modulus by > 40 to 80%, depending on the flame-retardant additive. The heat deflection temperature was reduced by about 30 to 60%, and the elongation at break was increased by 50 to 70% (Figure 5).
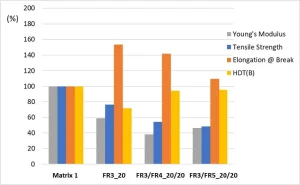
The addition of the solids FR4 and FR5 in 20 wt% content, respectively, together with 20 wt% FR3, resulted in a roughly 20% increase in heat deflection temperature, but the tensile properties were compromised significantly (Figure 6).
Conclusion
The investigated material strategy shows tremendous potential for the development of flame-retardant photopolymers via Hot Lithography for the 3D printing industry. The results highlight how flame resistance can be efficiently introduced into state-of-the-art methacrylate-based photopolymers for 3D printing by selection of industry-known flame-retardant additives, yet detailed experiments for the elucidation of possible synergistic effects and careful selection of formulation components to compensate for possible negative effects on printability and (thermo)mechanical performance need to be taken into consideration. Specifically, the new patented technology from the authoring company was implemented, giving rise to 1) unique performance photopolymers (e.g., materials with UL 94 V0 classification) and 2) industrially relevant production throughput and quality (e.g., Cerion production plant), thus enabling flexible production of functional AM parts optimized for the electronics industry.
For more information, contact Christian Gorsche at christian.gorsche@cubicure.com.
Acknowledgements
The authors gratefully acknowledge the financial support by the Austrian Research Promotion Agency (FFG, within the projects “3D HiPerPolymers” – number 874202 and “Polyflame” – number 870576).
References
- Wohlers Report 2020 – https://wohlersassociates.com/2020report.htm
- M. Pfaffinger, “Hot Lithography – New Possibilities in Polymer 3D Printing: A newly developed stereolithography-based additive manufacturing technology combines good material properties with outstanding manufacturing precision,” Laser Tech. J., 2018, 15 (4), 45–47.
- G. Peer, P. Dorfinger, T. Koch, J. Stampfl, C. Gorsche and R. Liska, “Photopolymerization of Cyclopolymerizable Monomers and Their Application in Hot Lithography,” Macromolecules, 2018, 51 (22), 9344-9353.